February 24, 2012 feature
Your brain on dye: Imaging neuronal voltage with fluorescent sensors and molecular wires
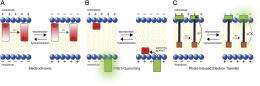
(Medical Xpress) -- Optically monitoring the brain’s neuronal activity can be accomplished in several ways, including electrochromic dyes, hydrophobic anions, calcium imaging, or voltage-sensitive ion channels. Fluorescence imaging is an attractive method due to its ability to map the electrical activity and communication of multiple spatially resolved neurons. While this complements traditional electrophysiological measurements, historically fluorescent voltage imaging has been limited by the difficulty of developing sensors that give both large and fast responses to voltage changes. Recently, however, scientists in the Department of Pharmacology and other areas in the University of California at San Diego’s Howard Hughes Medical Institute have designed, synthesized, and implemented fluorescent sensors in the form of photo-induced electron transfer (PeT)-based molecular wire probes for voltage imaging in neurons. Moreover, they have used these so-called VoltageFluor sensors to perform single-trial detection of synaptic and action potentials in cultured hippocampal neurons and intact leech ganglia.
The research team, led by post-doctoral researcher Evan W. Miller in the Tsien Laboratory, encountered a series of challenges in the design, synthesis, and application of PeT sensors, Miller tells Medical Xpress. “The main challenges we faced were designing and synthesizing a fluorescent sensor that would give both large and fast changes in fluorescence in response to voltage changes—something that had previously been difficult to accomplish. We also wanted something that would avoid placing a capacitative load on neurons,” he adds, “because this would limit our ability to watch normal brain activity.”
Their next challenge, Miller continues, was to think very carefully about localization. “For fluorescent voltage sensors, as opposed to calcium indicators, the probe must be localized to the membrane. That placed an additional constraint on our design, since we had to make a molecule which would be water-soluble enough to be compatible with live cell samples, but lipid-soluble enough to reside primarily in the plasma membrane.” While Miller thinks that they’ve addressed this problem to some extent with these molecules, he notes that there’s still a lot of room for improvement.
“Our final consideration was thinking about how to sense the entire transmembrane potential—in other words, how could we transfer an electron through a significant portion of the transmembrane electric field to sense a large fraction of the voltage change without losing electron transfer, or eT, efficiency?” This was critical because eT efficiency falls off exponentially as the distance between eT partners increases. To address the challenges that long-range eT presented—for example, they could sense more of the electric field by transferring an electron farther, but as a result would lose eT efficiency with distance—the team decided to try molecular wires as intervening spacers between electron donor and acceptor. “We thought this would enable long-range, distance-independent PeT that would be sensitive to external electric fields,” Miller notes. “We then had to determine how to synthesize this molecule.”
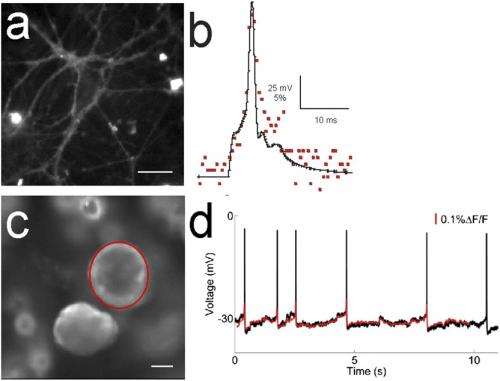
The scientists are already focused on other innovations that might be developed and applied to the current study. “We still have not stretched a molecular wire all the way across the membrane, which we believe will give us maximal voltage sensitivity,” Miller explains. “We’d also like a way to target this dye to genetically defined neurons, which should increase our signal-to-noise ratio—we wouldn’t be targeting non-excitable membranes, so this would allow us to make more sensitive measurements.”
Moreover, Miller notes, the team already has some derivatives in the pipeline that they hope will show improved sensitivity. “We also need to try and push the current derivatives and see what types of systems and preparations they can be useful in, beyond what we demonstrated in our initial publication. In addition, the probes can be optimized synthetically to achieve larger fluorescent changes per unit change in voltage.”
Even at this early stage, says Miller, the probes are already valuable indicators of neuronal activity. “One of the experiments I’m most excited about was the one we did in leech neurons with our collaborators in the Kristan lab at UCSD. We were able to show essentially a 1:1 correspondence between what would be recorded with an electrode—a more traditional method—compared with the fluorescence changes we measure optically with our dye. So, in an intact ganglia, which has several hundred neurons interconnected and firing, we can record the fluorescence changes and be confident that what we are seeing corresponds quite well to what we otherwise have to measure with an electrode. This allows us to make simultaneous measurements of multiple neurons in a circuit—something we couldn’t do with traditional electrophysiology.”
Miller also says that their method would be complementary to optogenetics that use light to control neurons—for example, Channelrhodopsin or Halorhodopsin systems. “Right now there is significant spectral overlap between the wavelengths that would need to be used for our dyes and the various light-gated channels,” he points out. “It would be nice to be able to use light to both stimulate neurons using optogenetic light-gated channels and then to read out the downstream ramifications of that using our fluorescent voltage sensors. However,” he adds, “we’d have to design some wavelength-shifted variants before that will be a possibility.”
Regarding other technologies or applications that might benefit from their findings, Miller tells PhysOrg that although they’ve focused primarily on the brain, potential applications include “any biological system in which voltage changes are important. One that comes readily to mind is the heart.”
More information: Optically monitoring voltage in neurons by photo-induced electron transfer through molecular wires, Published online before print January 24, 2012, doi: 10.1073/pnas.1120694109, PNAS February 7, 2012 vol. 109 no. 6 2114-2119.
Copyright 2012 Medical Xpress.
All rights reserved. This material may not be published, broadcast, rewritten or redistributed in whole or part without the express written permission of PhysOrg.com.